Journal of Musculoskeletal Disorders and Treatment
Effects of Seated Whole-Body Vibration Exposure on Cervical and Trunk Proprioception and Static and Dynamic Postural Stability
Takashi Nagai1*, Heather M Bansbach1, Mallory S Faherty1, John P Abt2, Timothy C Sell3 and Scott M Lephart2
1Neuromuscular Research Laboratory, University Of Pittsburgh, Pittsburgh
2Sports Medicine Research Institute, University Of Kentucky, Lexington
3Michael W Krzyzewski Human Performance Laboratory, Duke University, Durham
*Corresponding author:
Takashi Nagai, Ph.D., Neuromuscular Research Laboratory, University Of Pittsburgh, 3860 South Water Street, Pittsburgh, PA 15203, Tel: 412-246-0460, Fax: 412-246-0461, E-mail: tnagai@pitt.edu
J Musculoskelet Disord Treat, JMDT-2-024, (Volume 2, Issue 4), Research Article
Received: June 20, 2016; Accepted: October 03, 2016; Published: October 05, 2016
Citation: Nagai T, Bansbach HM, Faherty MS, Abt JP, Sell TC, et al. (2016) Effects of Seated Whole-Body Vibration Exposure on Cervical and Trunk Proprioception and Static and Dynamic Postural Stability. J Musculoskelet Disord Treat 2:024.
Copyright: © 2016 Nagai T, et al. This is an open-access article distributed under the terms of the Creative Commons Attribution License, which permits unrestricted use, distribution, and reproduction in any medium, provided the original author and source are credited.
Abstract
Introduction:Prolonged exposure to seated whole-body vibration (WBV) is considered a risk factor for neck pain (NP) and low back pain (LBP) in aircrew. Decreased trunk proprioception following WBV exposure has been previously reported. Proprioceptive feedback from the mechanoreceptors of the neck and trunk regions plays an important role in maintaining proper postural stability; therefore, it was hypothesized that WBV exposure would negatively affect both cervical/trunk proprioception and postural stability, potentially exposing aircrew to a greater risk of NP/LBP. The purpose of this study was to examine the effects of WBV on cervical and trunk proprioception and postural stability.
Methods:A total of 15 healthy subjects (6 males and 9 females) participated (age: 25.4 ± 4.5 years, height: 169.0 ± 7.8 cm, weight: 69.9 ± 10.9 kg). On three separate visits, subjects performed one of three testing protocols before and after seated WBV exposure for 30 minutes: 1. Cervical spine rotation joint position sense (JPS); 2. Trunk flexion JPS and trunk flexion/extension threshold to detect passive motion (TTDPM); and 3. Single leg eyes-open/closed static postural stability and dynamic postural stability. Cervical/trunk JPS was calculated as an absolute difference between the reference and replicated trials. Trunk TTDPM was calculated as time to perceive a passive movement and direction. The standard deviation of the ground reaction forces in anterior-posterior, medial-lateral, and vertical directions during a 10-second static balance was used for static postural stability. The first three seconds of the ground reaction forces during a forward jump-landing task were used to calculate dynamic postural stability index. Paired t-tests or Wilcoxon Signed Ranks tests were used to examine the difference before and after the seated WBV exposure across all variables. A Bonferroni correction was applied to the accepted p-value (p < 0.01).
Results:No significant changes were observed in cervical spine JPS, trunk JPS/TTDPM, or static/dynamic postural stability variables.
Discussion:Based on the current findings, the seated WBV exposure does not seem to influence proprioception and static/dynamic postural stability. Future studies should consider the occupational vibration, which has cumulative effects and other combined factors such as posture and muscular fatigue.
Keywords
WBV, Proprioception, Postural Stability
Introduction
A high prevalence of neck pain (NP) and low back pain (LBP) in military helicopter aircrew has been reported as high as 84.5% and 92%, respectively [1,2]. Furthermore, age and total flight-hours have been reported as contributing factors for NP/LBP, in part due to prolonged exposure to mechanical whole body vibration (WBV) and poor sitting posture in a confined cockpit [3,4]. It has been reported that WBV frequency during helicopter operation ranges from 1 to 10 Hz and the human body's resonance ranges from 3 to 7 Hz [5,6]. It is hypothesized that at the body's resonance, vibration transmission and amplitude magnifies throughout the spine, potentially causing premature muscular fatigue and compromised neuromuscular control [5,6] . The previous study has indicated an increase in muscle activation of the lumbar and thoracic spinal muscles during seated WBV exposure [7]. In turn, greater muscle activation must be met by increased metabolic/energy demand [8]. Overtime, muscular fatigue and associated changes in neuromuscular control may occur. For the same reasons (seated WBV exposure), other occupations such as large farm-machine operators and professional drivers are known to have NP and LBP issues [9,10] .
Seated WBV exposure is also thought to influence one's proprioceptive feedback by stimulating muscles spindles. The primary and secondary endings of muscle spindles contribute the sensation of joint position sense and movement (kinesthesia) while the secondary endings are mainly for joint position sense [11]. In earlier studies, muscle spindles are shown to be sensitive to high frequency vibration (80-150 Hz), and the application of vibration can result in overstimulation of the gamma motor neurons and false sensation of limb movement (even though there is no limb movement) [12]. Because proprioception provides information regarding joint position sense, kinesthesia, and sense of heaviness about joints [13] and plays an important role in the control of posture and locomotion [14], exposure to vibration can influence one's postural stability. Specifically, the proprioceptive information from neck muscle spindles influence head position, eye movement, and postural stability with the cervico-collic reflex, the cervico-ocular reflex, and the tonic neck reflex, respectively, during static and dynamic tasks [15]. The proprioceptive information from the lumbar spine muscles also influence one's lumbar spine joint position sense as muscle activities are altered through the application of vibration and corresponding distorted/biased proprioceptive signal for processing [16,17].
However, compared to an application of high frequency vibration to local postural muscles, the effects of seated WBV on proprioception and balance are not well understood. Previously, seated WBV exposure for 30 minutes negatively influenced balance during an unstable seated balance task [18]. Similarly, seated WBV exposure for 20 minutes resulted in increased trunk joint position sense errors [19]. On the other hand, a more recent study has reported that WBV did not alter joint position sense at the knee and ankle or static balance [20].
To our knowledge, few studies have evaluated the effects of seated WBV exposure (that simulated aircrew vibration) on both cervical/trunk proprioception and static/dynamic postural stability. Therefore, the purpose of the study was to evaluate the effects of seated WBV exposure on cervical/trunk proprioception and static/dynamic postural stability. It was hypothesized that after seated WBV exposure, subjects would exhibit poor cervical proprioception (joint position sense (JPS)), trunk proprioception (JPS and trunk flexion/extension threshold to detect passive motion (TTDPM)), and balance (single leg static and dynamic balance). It is clinically important to understand how cervical/trunk proprioception and static/dynamic postural stability could be altered after seated WBV exposure because diminished proprioception and balance are considered as risk factors of individuals with NP/LBP.
Methods
Study design and subjects
A total of 15 recreationally active adults (6 males and 9 females) participated in this study (age: 25.4 ± 4.5 years, height: 169.0 ± 7.8 cm, weight: 69.9 ± 10.9 kg). The number of subjects was determined using the power analysis (α = 0.05 and 1-β = 0.8) based on the previous results task [18]. Recreationally active is operationally defined as an individual who participates in physical activity at minimum of three times per week for at least 30 minutes per session. Individuals with aged 18 to 40 years were included in order to minimize age-related differences on proprioception and balance [21]. Individuals were excluded if currently experiencing NP or LBP, had a history of spine surgery or concussion, or their occupations involve WBV exposure (professional driver, farmers, aircrew, construction workers, etc). Prior to each test session, subjects were encouraged to avoid any unfamiliar exercises, heavy lifting, or endurance sports as fatigue and repetitive eccentric loading have shown to alter proprioception. Each subject visited the human performance laboratory three times at least one day apart. Subjects performed the following testing, each on a separate day: 1. Cervical rotation JPS; 2. Trunk flexion JPS and TTDPM; and 3. Single-leg static and dynamic postural stability. These tasks were completed before and immediately after a 30-minute seated WBV. WBV occurred with the subject in a seated position, directly on the VibePlate XL (VibePlate, Lincoln, NE). The VibePlate XL measures 76.2 cm by 182.9 cm, allowing for the subjects entire body to be on the VibePlate XL for the duration of the WBV. The order of modalities (cervical JPS, trunk JPS/TTDPM, and balance) was determined by a Latin-square method to eliminate any learning effects. Each visit took approximately one hour and 30 minutes. Prior to data collection, the protocol was reviewed and approved by the University of Pittsburgh Institutional Review Board (IRB).
Instrumentation and procedures
Cervical and trunk JPS were collected using a Vicon Nexus motion capture system synchronized with 10 MX13 infrared cameras (Vicon Motion Systems, Centennial, CO). Trunk TTDPM was collected using a BIODEX System 3 PRO dynamometer (BIODEX, Shirley, NY). Static and dynamic balance was collected using a Kistler force plate (Kistler 9286A, Amherst, NY). Seated WBV was administered while seated for 30 minutes using a low frequency vibration plate (VibePlate, Lincoln, NE) (frequency 10 Hz, oscillating amplitude: 2 mm).
For cervical JPS testing, left and right rotation JPS at angles of 30° (L30, R30) and 60° (L60, R60) were tested. Subjects were fitted with a helmet (HGU-56, Gentex Corporation, Simpson, PA), blindfolded, and seated on a wooden chair with hips and knees at approximately 90° flexion and feet hip-width apart. Fourteen millimeter diameter retroreflective markers were placed on both sides of the helmet (over the midline of the sphenoid bone (temple) and the most posterior aspect of the parietal bone without passing the inferior temporal line on the helmet). Additional markers were placed directly on the skin over C7, T10, the jugular notch, and xiphoid process. After a static capture trial, the subject practiced full left and right active rotation three times. The subject then performed a target angle trial followed by a reproduction angle trial (Figure 1). This was repeated six times for each direction and angle. Vicon Nexus Software was used to reconstruct position of the reflective markers in 3-dimensional space. Marker trajectories were smoothed using a general cross-validation Woltring filter and subsequently filtered using a fourth-order low-pass Butterworth filter with a 20 Hz cutoff frequency. Absolute values between a target angle and replicated angle were defined as JPS absolute error (AE). An average of six trials of JPS AE at each position (R30, L30, R60, and L60) was used for statistical analyses. Reliability and precision of the cervical JPS tests was previously established in our laboratory (Intraclass Correlation Coefficient (ICC) = 0.44-0.81; Standard Error of Measurement (SEM) = 0.64-1.28 degrees) [22].
For trunk JPS testing, markers were be placed on the following anatomical landmarks: 2nd metatarsal head (dorsal aspect), lateral malleolus (distal tip), posterior calcaneus (at the level of the 2nd metatarsal marker), lateral aspect of lower leg (midpoint between lateral malleolus and lateral femoral condyle markers), lateral femoral epicondyle, lateral aspect of the upper leg (midpoint between lateral femoral condyle marker and the greater trochanter of the femur), anterior superior iliac spine, posterior superior iliac spine, T10, C7, xiphoid process, and, jugular notch. After a static capture, participants were instructed to stand upright with weight equally distributed on both feet near the center of the capture area. A custom guide was placed in front of the subject so that the crossbar contacts the anterior thigh. A subject was asked to forward flex 20 degrees until the anterior abdomen contacted the guide and press a trigger to mark the position. Then, the subject returned to the start position and attempted to replicate the target position without a guide (Figure 1). This test was repeated six times. Marker trajectories were reconstructed, smoothed, and filtered similar to the cervical JPS AE. An average of six trials of trunk JPS AE was used for statistical analyses. Reliability and precision of the trunk JPS tests was previously established in our laboratory (ICC = 0.84 - 0.98; SEM = 0.2 - 1.0 degrees) [23].
For trunk flexion-extension TTDPM testing, a subject wore a blindfold and noise cancelling headphones. The subject was seated on the Biodex and strapped with two Velcro straps across the thighs and pelvis to minimize hip involvement, and the H-harness across the chest very firmly to ensure the trunk moved consistently with the dynamometer (Figure 1). The Biodex was initiated to passively move at 0.25 degrees per second. The investigator instructed the subject to press a hold button upon first perception of trunk motion and identify in which direction the movement occurred. If movement direction was identified incorrectly, that trial was discarded. The time from first instruction to commencement of motion was selected by the investigator between 3 - 20 seconds. A total of 12 trials were performed, six toward flexion and six toward extension in random order. The TTDPM was calculated as the difference between the angle of detection and the starting angle. An average of six trials in each direction was averaged and used for statistical analyses. Reliability and precision of TTDPM were previously established in our laboratory (ICC = 0.90; SEM = 0.05 degrees) [24].
For balance testing, a subject performed three trials (10 seconds each) of a single-leg standing balance test (barefooted) under eyes-open and eyes-closed conditions. The subject was asked to remain as still as possible with the non-stance limb raised to about the level of the stance limb ankle without touching the stance limb and with hands on their hips (Figure 2). The subject was permitted to touch down on the force plate with the non-stance limb during the trial [25]. However, if a subject stepped off of the force plate during the 10 second trial, those trials were repeated. Standard deviations of ground reaction forces in three planes (anterior-posterior, medial-lateral, and vertical) during the 10-second trial were calculated and used for statistical analyses. The dominant leg, defined as a preferred leg to kick a ball, was analyzed. Reliability and precision of single-leg balance testing was previously established in our laboratory (ICC = 0.71 - 0.94; SEM = 0.19 - 3.40 Newtons) [26].
For dynamic balance, a subject was asked to stand on two legs at a distance of 40% of his or her body height from the force plate. The subject was asked to jump forward on to the force plate, initiating the jump from two limbs and clearing a 12-inch hurdle, which was placed at the midpoint between the subjects's starting spot and the force plate. The subject was asked to land on their dominant leg. Upon landing, the subject was asked to stabilize as quickly as possible, place hands on hips, and balance for 10 seconds (Figure 3). Trials were discarded and re-assessed if the non-dominant leg touched the dominant leg or the ground. Trials were also discarded if the subject's dominant heel shifted upon landing or if a bounce occurred after initial contact with the force plate. Dynamic postural stability index in the anterior-posterior, medial-lateral, vertical, and combined directions, were calculated root mean square deviations about a zero point during the first three seconds of landing after initial contact with the force plate and normalized to body weight [26]. Initial contact was defined as the point where the vertical ground reaction force exceeded 5% of the participant's body weight. An average of three trials was used for statistical analyses. Reliability and precision of dynamic postural stability testing was previously established in our laboratory (ICC = 0.86; SEM = 0.001) [26].
Statistical analyses
Descriptive statistics (means and standard deviations) were calculated for all dependent variables before and after WBV exposure for 30 minutes (pre/post). Each dependent variable was checked for normality using Shapiro-Wilk tests. Based on the normality, paired t-test or nonparametric Wilcoxon signed rank test were used to compare neck and trunk proprioception and balance before and after seated WBV. Effect size (Cohen's d) was calculated based on the means and standard deviations of pre/post values [27]. Significance was adjusted for multiple comparisons with Bonferroni Correction (p < 0.01 a priori). All statistical analyses were performed using SPSS version 20.0 (IBM Corporation, Armonk, NY).
Results
Descriptive statistics are represented in table 1. There were no statistically significant differences in Cervical JPS AE (p = 0.064-0.411). Similarly, trunk JPS AE and trunk TTDPM were not statistically significant (p = 0.029-1.000). For static postural stability, there were no statistically significant differences in all variables both EO and EC conditions (p = 0.068-0.427). Similarly, dynamic postural stability variables were not statistically different (p = 0.113-0.820).
Table 1: Proprioception and Balance Results Pre- and Post-WBV Exposure (means ± standard deviations).
View Table 1
Discussion
The current study evaluated the effects of WBV on cervical/trunk proprioception and static/dynamic postural stability. It was hypothesized that after seated WBV exposure, there would be significant reductions in cervical/trunk proprioception and balance. Contrary to the hypotheses, none of variables were significantly different after the seated WBV exposure. Therefore, based on the current findings, seated WBV exposure does not negatively influence cervical/trunk JPS AE, trunk TTDPM, and postural stability. From a methodological perspective, all values of cervical/trunk JPS AE, trunk TTDPM, and postural stability were similar to previous studies with the same procedures [22-24,26]. The current findings are also clinically important. Each dependent variable is further discussed below.
Cervical proprioception has received a significant amount of attention due to its influence on balance, perception of motion, and motor control [28]. In short, cervical proprioceptive and vestibular input converges and interacts at the vestibular nuclei, the cerebellum, and the parieto-insular vestibular cortex, playing an essential role in conscious awareness of motion, spatial orientation, and body motion [28]. Cervical proprioception is commonly evaluated using either the head-to-neutral reposition test or JPS. In the current study, cervical proprioception was measured using JPS, and there was no significant change before and after seat WBV exposure. A recent meta-analysis revealed that the head-to-neutral reposition test was able to differentiate individuals with and without NP [29]. Based on this meta-analysis on cervical proprioception methodologies, it is speculated that JPS may not be sensitive enough to detect small differences caused by seated WBV exposure. There were very few studies examining the effects of WBV on cervical proprioception to compare with the current study. More recently, Beinerd, et al. [30] compared individuals with and without NP before and after an application of handheld high frequency vibration (100 Hz, 1 mm amplitude) to the neck region. The authors reported a significant reduction in JPS error (better proprioception) in subjects with NP while a significant increase in JPS error (worse proprioception) in subjects without NP was observed [30]. More research is needed to understand the effects of seated WBV exposure to cervical proprioception.
As to trunk proprioception, there were no significant differences after seated WBV exposure. Previously, there were mixed results on the effects of WBV on trunk proprioception [19,31,32]. A study by Li, et al. [19] evaluated trunk JPS before and after 20-min WBV exposure (5.0 Hz, 0.22 m/s2) and indicated 1.58-fold increase in trunk JPS error. Similarly, a study by Lee and Chow [31] evaluated trunk JPS before and after 5-min WBV exposure (18.0 Hz, 6 mm amplitude); however, there were no significant changes on trunk JPS. A study by Fontana, et al. [32] used 5-min WBV exposure (18.0 Hz, 10 mm amplitude) in semi-squat position and found a significant reduction in trunk JPS error (better JPS) after the exposure, suggesting a positive influence of WBV on trunk JPS. The results of the current study were in accordance with the study by Fontana, et al. [32]. Interestingly, similar to the cervical JPS studies in individuals with NP [30], it has been reported that applying handheld vibration (70.0 Hz, 0.5 mm amplitude) on the paraspinal muscles actually reduces trunk JPS error (better proprioception) in individuals with LBP [17]. It is beyond the scope of the current investigation; however, future investigations should explore potential use of vibration as a treatment of NP and LBP. In fact, use of vibration as a part of neurorehabilitation for individuals with neurological conditions: spinal cord injury, multiple sclerosis, and Parkinson's disease have been used to reduce spasticity and promote motor activity and locomotion [33].
The current investigation revealed that there were no effects of WBV on static and dynamic postural stability. This finding was in accordance with the previous studies, which found no effect of WBV on static and dynamic postural stability [20,34]. Pollock, et al. [20] examined static postural stability eyes-open and eyes-closed before and after a 5-minute (1 minute × 5) WBV exposure (30 Hz, 4-8 mm amplitude) finding no significant changes [20]. Interestingly, the authors also examined 15 minutes and 30 minutes after the WBV exposure and found that the variation of the vertical component of the center of mass acceleration after 30 minutes was actually better than the baseline values in the eyes-open conditions [20]. Similarly, Torvinen, et al. [34] evaluated the effects of a 4-minute WBV exposure (25.0-40.0 Hz, 2 mm amplitude) on performance tests (maximal leg press, vertical jump, grip strength, and shuttle run) and balance tests (Biodex Stability System (BIODEX, Shirley, NY) postural sway test and tandem walk test), reporting no significant changes on those parameters. Contrarily, a higher frequency (44 Hz) WBV exposure has shown to decrease postural stability, likely greater activation of mechanoreceptors [35]. It is also important to recognize that the inconsistency of findings in literature might be explained by the methodological differences between the studies, related to both the vibration parameters and the measures assessed.
There have been few studies examining the effects of WBV on dynamic postural stability. As previously stated, Beinert, et al. [30] used a perturbation platform to evaluate dynamic balance before and after hand-held high frequency vibration to neck muscles. They reported significant reduction in sway distance (better dynamic postural stability) in individuals with NP following the application of the hand-held high frequency vibration [30]. With these previous results and the results of the current study, it could be concluded that minimal changes on static and dynamic postural stability can be expected after seated WBV exposure.
There are limitations in the current investigation. We did not utilize accelerometers to actually see how much vibration subjects were experiencing at each level of the spine (lumbar, thoracic, cervical, and head) while sitting on the WBV plate. We also did not evaluate muscular electromyography to observe muscle activities in the spine and potential fatigue effects using frequency analyses. Another limitation is a lack of generalizability. Sitting on the WBV plate for 30 minutes is not the same as riding on the helicopter for several hours with full combat gears in their career (cumulative effects). Similar investigations should be conducted under real occupational settings.
In conclusion, to our knowledge, the present study is the first to evaluate the effects of seated WBV exposure on cervical/trunk proprioception and static/dynamic postural stability. The results indicate that the seated WBV exposure may have minimal to no negative effect on those characteristics. Instead, positive effects of vibration have been reported: applications of high frequency vibration have been used in the neurorehabilitation [33] and have shown to improve kinesthesia and balance [36]. A recent review paper on WBV studies also supports this contention [37]. Another study has shown positive effects of WBV on a functional task in untrained elderly subjects [38]. However, we should interpret the current results cautiously as there have been few studies evaluating chronic effects (over their career) of seated WBV exposure on cervical/trunk proprioception and balance and the development of neurological/musculoskeletal conditions in aircrew. It is important to recognize that this topic (effects of occupational WBV) is very complex as the effects of WBV might be dependent on several factors, such as the parameters of stimulation (frequency, duration, amplitude and direction), the position of the subjects during vibration, the place of stimulation. Additionally, given the importance of cervical/trunk proprioception and its role in balance, spatial orientation, and motor control, future studies should include the vestibular and visual system and evaluate how each system interacts with WBV.
References
-
Salmon DM, Harrison MF, Neary JP (2011) Neck pain in military helicopter aircrew and the role of exercise therapy. Aviat Space Environ Med 82: 978-987.
-
Gaydos SJ (2012) Low back pain: considerations for rotary-wing aircrew. Aviat Space Environ Med 83: 879-889.
-
van den Oord MH, De Loose V, Meeuwsen T, Sluiter JK, Frings-Dresen MH (2010) Neck pain in military helicopter pilots: prevalence and associated factors. Mil Med 175: 55-60.
-
Bongers PM, Hulshof CT, Dijkstra L, Boshuizen HC, Groenhout HJ, et al. (1990) Back pain and exposure to whole body vibration in helicopter pilots. Ergonomics 33: 1007-1026.
-
Kitazaki S, Griffin MJ (1998) Resonance behaviour of the seated human body and effects of posture. J Biomech 31: 143-149.
-
De Oliveira CG, Nadal J (2005) Transmissibility of helicopter vibration in the spines of pilots in flight. Aviat Space Environ Med 76: 576-580.
-
Baig HA, Dorman DB, Bulka BA, Shivers BL, Chancey VC, et al. (2014) Characterization of the frequency and muscle responses of the lumbar and thoracic spines of seated volunteers during sinusoidal whole body vibration. J Biomech Eng 136: 101002.
-
Games KE, Sefton JM, Wilson AE (2015) Whole-body vibration and blood flow and muscle oxygenation: a meta-analysis. J Athl Train 50: 542-549.
-
Milosavljevic S, Bagheri N, Vasiljev RM, McBride DI, Rehn B (2012) Does daily exposure to whole-body vibration and mechanical shock relate to the prevalence of low back and neck pain in a rural workforce? Ann Occup Hyg 56:10-17.
-
Bovenzi M (2009) Metrics of whole-body vibration and exposure-response relationship for low back pain in professional drivers: a prospective cohort study. Int Arch Occup Environ Health 82: 893-917.
-
Proske U, Gandevia SC (2009) The kinaesthetic senses. J Physiol 587: 4139-4146.
-
Goodwin GM, McCloskey DI, Matthews PB (1972) The contribution of muscle afferents to kinaesthesia shown by vibration induced illusions of movement and by the effects of paralysing joint afferents. Brain 95: 705-748.
-
Lephart SM, Riemann BL, Fu FH (2000) Introduction to the sensorimotor system. In: Lephart SM, Fu FH, editors, Proprioception and Neuromuscular Control in Joint Stability. Champaign, IL: Human Kinetics xvii-xxiv.
-
Courtine G, De Nunzio AM, Schmid M, Beretta MV, Schieppati M (2007) Stance- and locomotion-dependent processing of vibration-induced proprioceptive inflow from multiple muscles in humans. J Neurophysiol 97: 772-779.
-
Treleaven J (2008) Sensorimotor disturbances in neck disorders affecting postural stability, head and eye movement control. Man Ther 13: 2-11.
-
Brumagne S, Lysens R, Swinnen S, Verschueren S (1999) Effect of paraspinal muscle vibration on position sense of the lumbosacral spine. Spine (Phila Pa 1976) 24: 1328-1331.
-
Brumagne S, Cordo P, Lysens R, Verschueren S, Swinnen S (2000) The role of paraspinal muscle spindles in lumbosacral position sense in individuals with and without low back pain. Spine (Phila Pa 1976) 25: 989-994.
-
Slota GP, Granata KP, Madigan ML (2008) Effects of seated whole-body vibration on postural control of the trunk during unstable seated balance. Clin Biomech (Bristol, Avon) 23: 381-386.
-
Li L, Lamis F, Wilson SE (2008) Whole-body vibration alters proprioception in the trunk. Int J Ind Ergon 38: 792-800.
-
Pollock RD, Provan S, Martin FC, Newham DJ (2011) The effects of whole body vibration on balance, joint position sense and cutaneous sensation. Eur J Appl Physiol 111: 3069-3077.
-
Cohen H, Heaton LG, Congdon SL, Jenkins HA (1996) Changes in sensory organization test scores with age. Age Ageing 25: 39-44.
-
Nagai T, Abt JP, Sell TC, Clark NC, Smalley BW, et al. (2014) Neck proprioception, strength, flexibility, and posture in pilots with and without neck pain history. Aviat Space Environ Med 85: 529-535.
-
Tsai YS, Sell TC, Smoliga JM, Myers JB, Learman KE, et al. (2010) A comparison of physical characteristics and swing mechanics between golfers with and without a history of low back pain. J Orthop Sports Phys Ther 40: 430-438.
-
Learman KE, Myers JB, Lephart SM, Sell TC, Kerns GJ, et al. (2009) Effects of spinal manipulation on trunk proprioception in subjects with chronic low back pain during symptom remission. J Manipulative Physiol Ther 32: 118-126.
-
Goldie PA, Evans OM, Bach TM (1992) Steadiness in one-legged stance: development of a reliable force-platform testing procedure. Arch Phys Med Rehabil 73: 348-354.
-
Sell TC, House AJ, Abt JP, Huang HC, Lephart SM (2012) An examination, correlation, and comparison of static and dynamic measures of postural stability in healthy, physically active adults. Phys Ther Sport 13: 80-86.
-
Cohen J (1988) Statistical Power Analysis for the Behavioral Sciences. (2nd edn), Hillsdale, NJ: Lawrence Erlbaum Associates.
-
Pettorossi VE, Schieppati M (2014) Neck Proprioception Shapes Body Orientation and Perception of Motion. Front Hum Neurosci 8: 895.
-
Stanton TR, Leake HB, Chalmers KJ, Moseley GL (2016) Evidence of Impaired Proprioception in Chronic, Idiopathic Neck Pain: Systematic Review and Meta-Analysis. Phys Ther 96: 876-887.
-
Beinert K, Keller M, Taube W (2015) Neck muscle vibration can improve sensorimotor function in patients with neck pain. Spine J 15: 514-521.
-
Lee TY, Chow DH (2013) Effects of whole body vibration on spinal proprioception in normal individuals. Conference proceedings: Annual International Conference of the IEEE Engineering in Medicine and Biology Society. 2013: 4989-4992.
-
Fontana TL, Richardson CA, Stanton WR (2005) The effect of weight-bearing exercise with low frequency, whole body vibration on lumbosacral proprioception: a pilot study on normal subjects. Aust J Physiother 51: 259-263.
-
Murillo N, Valls-Sole J, Vidal J, Opisso E, Medina J, et al. (2014) Focal vibration in neurorehabilitation. Eur J Phys Rehabil Med 50: 231-242.
-
Torvinen S, Sievanen H, Jarvinen TA, Pasanen M, Kontulainen S, et al. (2002) Effect of 4-min vertical whole body vibration on muscle performance and body balance: a randomized cross-over study. Int J Sports Med 23: 374-379.
-
Sonza A, Robinson CC, Achaval M, Zaro MA (2015) Whole body vibration at different exposure frequencies: infrared thermography and physiological effects. ScientificWorld Journal 2015: 452657.
-
Ribot-Ciscar E, Hospod V, Aimonetti JM (2013) Noise-enhanced kinaesthesia: a psychophysical and microneurographic study. Exp Brain Res 228: 503-511.
-
Costantino C, Gimigliano R, Olvirri S, Gimigliano F (2014) Whole body vibration in sport: a critical review. J Sports Med Phys Fitness 54: 757-764.
-
Rogan S, Radlinger L, Hilfiker R, Schmidtbleicher D, de Bie RA, et al. (2015) Feasibility and effects of applying stochastic resonance whole-body vibration on untrained elderly: a randomized crossover pilot study. BMC Geriatr 15: 25.
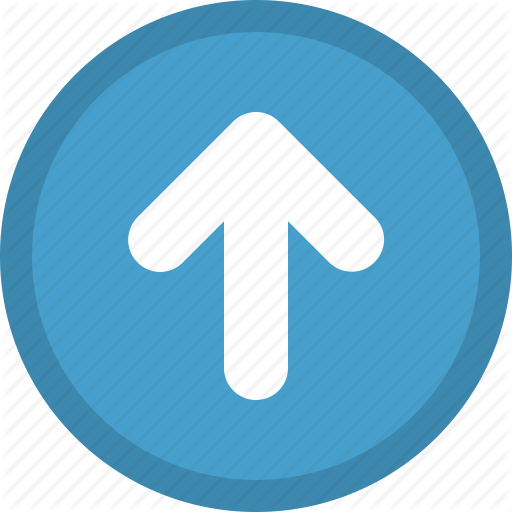